Global warming refers to increasing average Earth temperature caused by greenhouse gas emissions into the atmosphere. Currently, global warming is an important issue that concerns the world. It significantly impacts climate and the environment, including rising global average temperatures, alteration in extreme weather, and increasing sea level, raising global concerns over its impact on human life and ecosystems. According to the Indonesian Meteorological, Climatological, and Geophysical Agency, one such impact can be observed in Indonesia, where an average temperature in April 2023 showed a positive anomaly with a value of 0.2 °C.
On a global scale, according to the National Oceanic and Atmospheric Administration (NOAA) website, April 2023 is the fourth warmest month in the world in NOAA's 174-year history. Therefore, it is necessary to address climate change and support one of the goals of the Sustainable Development (SDGs) program, namely, tackling climate change.
One of the contributors to carbon emissions is animal waste, and one of them is manure. Animal manure contributes approximately 10% of carbon emissions to the total emissions, which includes CO2, CH4, and N2O [1]. Up to 2020, only 11% of biomass was used as a renewable energy source [2]. Similarly, just 1.64% of animal waste and 4.3% of biomass are renewable energy sources in Indonesia [3]. One of the benefits of using biomass as a sustainable energy source is that it grows quickly and is abundant. The quantity of manure produced by livestock such as sheep, goats, and cattle can vary depending on feed type, age, body weight, and animal health. Animal waste generally exists in solid, liquid, and gas forms.
Solid manure, including sheep and goat manure, typically has a lower moisture content of around 70–80%. Meanwhile, cow manure usually has a higher moisture content of around 85–90%. The composition of solid manure primarily consists of organic materials such as fibre, protein, and carbohydrates that are not digested by the livestock's digestive system. Sheep and goat solid manure contain more nitrogen and phosphorus than cow manure. The amount of manure produced by sheep weighing 20–40 kg is approximately 0.32 to 0.625 kg per day, equivalent to 0.3 tons per year [4]. The potential for renewable energy sources from biomass will increase in line with the growth of the livestock population [5]. Sheep manure contains a higher volatile content (58–64%) than cow manure (53%). Consequently, when it is converted through pyrolysis, sheep manure can produce higher biochar and syngas yield than cow manure. Furthermore, when compared to other manures, sheep manure has a higher fixed carbon content (approximately 13%), implying a greater calorific value [6].
Methods used to convert biomass energy are gasification, pyrolysis, and combustion. The fuel can be oxidised, and its energy content is transformed into heat during combustion. However, the efficiency of this combustion process is merely 10%, leading to environmental pollution as a consequence [4]. On the other hand, gasification involves a partial oxidation process of biomass and biomass conversion into fuel in gas. Pyrolysis is a thermal decomposition process using thermal energy with little or no oxygen [7]. The main advantage of pyrolysis is its adjustability according to the desired results.
To thoroughly comprehend biomass pyrolysis, it is essential to comprehend the thermal performance of the feedstock and its components [8]. The conversion of biomass into high-value products relies heavily on the rate of pyrolysis kinetics [9]. Further study of biomass properties requires analysis of Arrhenius parameters. The Arrhenius parameters of the thermal degradation process are essential for design optimisation, understanding reaction mechanisms, and predicting thermodynamic features for effective biomass energy production [8]. Determining the Arrhenius parameters involves conducting measurements and thermal analysis of biomass in a controlled environment, which can be achieved through the thermogravimetric analysis (TGA) technique. The TGA test results provide information about the extent of thermal degradation of biomass at various temperatures. By obtaining Arrhenius parameters, such as reaction rate constant, activation energy, and frequency factor, it is also possible to understand the kinetics of biomass pyrolysis [10]. It is crucial for designing efficient pyrolysis processes, understanding the involved reaction mechanism, and predicting the relevant thermodynamic properties for efficient energy generation from biomass.
Moreover, TGA is widely employed for studying pyrolysis kinetics, particularly in conditions with low heating rates, as it offers high precision [11]. Pyrolysis kinetics modelling can be achieved through three model approaches: two-stage semi-global, one-stage multiple reactions, and one-stage global single reaction [12].
Previously, several studies had been carried out on reaction kinetic analysis for several animal wastes. Chong et al. studies on reaction kinetic analysis of horse manure revealed promising parameters for modelling devolatilisation and designing thermochemical conversion processes, indicating its potential for bioenergy recovery [13]. Yuan et al. investigated the multi-stage nature of the cattle manure pyrolysis process, characterised by interactions between various components, elucidated through thermogravimetric analysis and isoconversional methods, providing valuable insights for reactor design and understanding of the pyrolysis mechanism [14].
The study conducted by Pu et al. elucidated the thermal decomposition characteristics and kinetics of chicken manure, revealing significant differences between nitrogen and air atmospheres. The optimal thermal decomposition was achieved under a 10 °C/min heating rate, with distinctive activation energies observed under different atmospheric conditions [15]. Conversely, Yıldız et al. utilised operating conditions with a heating rate ranging from 5 to 20 °C/min [10]. Parthasarathy et al. concluded that the study on the sustainable valorisation of camel manure through thermogravimetric analysis provided valuable insights into the kinetics and thermodynamic properties of O2 gasification, which can inform the design and optimisation of gasifiers for efficient utilisation of this waste resource [16]. Conversely, their studies on the pyrolytic behaviour of camel manure elucidated the effects of heating rates on thermal characteristics and char yield. However, it showed an insignificant impact on the kinetic parameters, providing valuable insights for designing pyrolysis reactors [17]. Apart from that, Ashraf et al. explored and compared the pyrolytic conversion of the dairy manure of two common milch animals (cow and buffalo) within confined animal feeding operations through detailed kinetic and thermodynamic analysis [18]. Wang et al. concluded that optimal performance of pyrolysis made from swine manure can be achieved at a pyrolysis temperature of 550 °C and a reaction time of 1 second [19]. Furthermore, Fernandez et al. studied swine manure and revealed consistent kinetics of pyrolysis and combustion processes, with the distributed activation energy model proving to be the most accurate for predicting weight loss curves [20]. However, to our knowledge, there has been no study on the reaction kinetics of pure sheep manure (without mixture). Research conducted by Akyürek (2021) analysed the reaction kinetics in the co-pyrolysis of sheep manure with recycled polyethylene terephthalate [23]. Focusing on sheep manure allows kinetic research to understand the reaction mechanism more profoundly. This includes determining the activation energy, reaction rate, and phase changes that occur during the pyrolysis process. A better understanding of the kinetics of these reactions is essential for optimising pyrolysis process parameters and increasing the efficiency of converting feedstocks into desired products.
Many TGA studies have been carried out on animal waste. Erdogdu et al. (2018) observed through their TGA analysis that the maximum separation rate occurred in the temperature range of 250 °C to 500 °C, with the complete conversion being achieved at approximately 600 °C [4]. Parthasarathy et al. (2022) conducted a study exploring kinetic models' bio-energy potential. This investigation involved the assessment of their physicochemical attributes, pyrolysis characteristics, and kinetic behaviour through thermodynamic analysis [21]. Martinez et al. (2020) investigated the slow pyrolysis of digested dairy cattle manure using one-step and multi-step pyrolysis processes, spanning a temperature range from 250 °C to 600 °C [22]. Rumaihi et al. (2021) compared pyrolysis kinetics with gasification kinetics and found that camel dung necessitates a higher activation energy for its pyrolysis decomposition [31]. Akyürek et al. (2021) investigated the synergetic effects of co-pyrolysis of different waste feedstocks, using the Flynn-Wall-Ozawa model to determine kinetic parameters, revealing that the apparent activation energy values for the decomposition of sheep manure into a recycled polyethylene terephthalate blend for green energy production [23]. However, that research has not included kinetic analysis of pure sheep manure using the Friedman model.
Consequently, research on the TGA of sheep manure is still limited. Therefore, kinetic and thermodynamic analyses were performed in this work using the Friedman and Coats-Redfern models on sheep manure. This research is significant for understanding animal manure biomass's thermal behaviour and degradation kinetics. The TGA method allows measuring changes in the mass of samples when heated in stages, thus providing insights into the thermal reactions. This research is expected to show that sheep manure's pyrolysis behaviour and thermal characteristics can be beneficial for developing sheep manure biomass utilisation.
This section describes the properties of feedstock pyrolysis and methods for analysing reaction kinetics.
This study was conducted in West Java, Indonesia. The pyrolysis used sheep manure as feedstock. The sheep manure was obtained from local farms in West Java, Indonesia. Based on data from the Indonesian Directorate of Livestock, Fisheries and Forestry Statistics, the sheep population has the greatest growth. The sheep population in Indonesia is experiencing positive growth, with an average annual growth rate of 0.97%, compared to other livestock populations, e.g. goats, cows, horses and pigs. Thus, it can be concluded that sheep manure has a high potential for usage as renewable biomass energy. Regionally, the sheep population is clustered in Java. West Java has the largest sheep population in Indonesia, with as many as 12.25 million heads. Therefore, West Java has a role in contributing almost 68.41% of the total sheep population in Indonesia [5].
Biomass analysis was carried out in this study through Simultaneous Thermogravimetric Analysis, Differential Thermal Analysis, and Differential Scanning Calorimetry (TGA-DTA-DSC) using NEXTA STA (Hitachi STA200RV with Real View Sample Observation). The inert gas used in this test is pure nitrogen. The used sample was sheep manure powder 6.790 mg. Before thermogravimetric testing, sheep manure was first separated from soil and other impurities. Furthermore, the sheep manure was dried until it reached a mass reduction of approximately 90%. A 100 ml/min nitrogen flow rate was used, and the sample was heated at a heating rate of 10 °C/min to 900 °C. TGA was used to study the degradation in the mass of the biomass sample as it was subjected to controlled heating. DSC measured the heat transformed during heating into enthalpy, while DTA measured the difference in sample temperature and standard (inert) material.
The TGA results were analysed using the Arrhenius equation with a one-stage global single reactions kinetics model. It is the simplest model, and it assumes that the decomposition rate depends on the reaction order as shown in eq. (1) [8], [21], [24]. Many studies on the analysis of pyrolysis kinetics use the TGA test results. There are three types of kinetic models used for the analysis of biomass pyrolysis: differential, isoconventional (model-free), model-fitting and pseudo-component [25]-[27]. The basic equation used in all studies on kinetics is shown in eq. (1) [28]:
(1)
where k(T) is the rate constant whose dimension for a first-order reaction is [1/s], T is temperature [K], and f(X) is a reaction model that describes the reaction mechanism. Conversion fraction X is shown in eq. (2):
(2)
To calculate the constant k, the Arrhenius equation, eq. (3), is used:
(3)
where A is the frequency factor [1/s], E is activation energy [J/mol], and R is the ideal gas constant equal to 8.314 J/(mol K).
From eq. (1) and eq. (3), eq. (4) is obtained:
(4)
If the temperature is varied by the heating rate , the Friedman kinetic model, eq. (5), is obtained:
(5)
Eq. (5) converted to logarithmic formula becomes eq. (6):
(6)
If the equation form y=a+bx is assumed, where x = 1/T, then:
(7)
(8)
(9)
The activation energy can be determined by plotting the experimental data with y on the vertical axis and x, that is, 1/T, on the horizontal axis. The slope of the plot yields the value of b, while the intercept provides the value of a.
The understanding of the kinetics of pyrolysis in sheep manure is reached by carrying out iso-conventional modelling, one of which is the Coats-Redfern model. First, the Friedman kinetic model focuses on the effect of the heating rate on the reaction process. It is useful for understanding how the decomposition rate of a substance changes with different heating rates because it produces kinetic parameters such as activation energy E and frequency factor A. On the other hand, the Coats-Redfern model provides a broader perspective by analysing Thermogravimetric (TG) and Differential Scanning Calorimetry (DSC) data to consider various reaction mechanisms possibly involved in the thermal degradation process. The Coats & Redfern method also called the model fitting method, falls into the category of single heating rate methods. Only one test with a certain TGA heating rate is needed, and then the kinetic parameter values can be known. In addition to the activation energy value and frequency factor, the Coats-Redfern method is also used to determine the thermal decomposition pattern of a material [29]. The fundamental equation for the Coats and Redfern method is given in eq. (10) [30]:
(10)
where f(X) is a kinetic function of the reaction mechanism.
Analyses of the thermodynamic parameters of the pyrolysis process are necessary to determine the reaction energy and stability. These thermodynamic parameters are calculated based on the results obtained from the reaction kinetics [21]. Rumaihi et al. derived estimates for activation energy values and applied the Kissinger equation to ascertain thermodynamic properties such as Gibbs free energy, enthalpy, and entropy [31]. This approach is similar to the research by Guo et al. on the pyrolysis of corn and cotton stalks, which calculated thermodynamic parameters [32]. Three key thermodynamic parameters must be computed for the pyrolysis process: enthalpy, entropy, and Gibbs free energy. These parameters play a crucial role in understanding the energy changes and feasibility of the pyrolysis reaction by providing important information on the spontaneity and directionality of the pyrolysis reaction, which is essential in determining its viability and potential applications.
Enthalpy H describes the heat energy released or absorbed during a pyrolysis reaction. In a pyrolysis system, enthalpy is the energy required to increase the raw material's temperature from room to the reaction temperature to convert biomass into gas, char, and liquid pyrolysis products [33]. The measurement of enthalpy change ΔH is crucial for understanding the thermal characteristics of the biomass pyrolysis process. A negative ΔH value indicates that the pyrolysis reaction released heat energy, while a positive ΔH value indicates that heat energy is absorbed during the reaction.
The equation for the enthalpy change is shown in eq. (11). By accurately determining the enthalpy change, valuable insights can be gained about the energy balance and efficiency of pyrolysis processes, which are important for optimising and designing pyrolysis systems for various applications.
(11)
where TP [K] denotes temperature at the peak of sample decomposition.
Gibbs free energy G shows the thermodynamic energy of a system that can be converted into work at constant T and P. The Gibbs free energy reaches its maximum value when the process is reversible. The equation for ΔG is shown in eq. (12).
(12)
where kB is the Boltzmann constant equal to 1.38 ×10−23 J/K and h is the Planck constant equal to 6.63 ×10−34 J s.
Entropy S is a thermodynamic parameter that measures the degree of disorder or randomness in a system. The relationship for ΔS is shown in eq. (13).
(13)
This section presents the findings derived from each step of the evaluation.
The proximate and ultimate test results on the sheep manure sample used in this study are shown in Table 1. The main characteristics of sheep manure are shown in moisture content, proximate test results (ash, volatile matter and fixed carbon) and ultimate analysis (C, H, N, O, S). Several components that can affect the yield of pyrolysis products are moisture, ash, sulphur and nitrogen [34]. Biomass with high moisture content requires a large energy input for the raw material pretreatment, especially for the raw material drying. High ash content can increase biochar yield [35], [36] but decrease bio-oil yield [34]. High ash content in biomass can increase the selling price of fuel, while a high O/C ratio can reduce the selling price of fuel in biomass with the same ash content [34]. High volatile matter content in biomass can also reduce biochar yields [37].
Comparison of sheep manure properties [%]
Property | As-received basis [44] | As-received basis [6] | Dry basis [34] | Dry basis (current study) |
---|---|---|---|---|
Ultimate | ||||
C | 49.0 ± 0.5 | 51.33 | 34.33 | 33.97 |
H | 6.3 ± 0.1 | 6.45 | 4.96 | 4.27 |
N | 3.3 ± 0.0 | 2.65 | 2.36 | 3.37 |
O | 41.3 | 38.81 | 41.96 | 25.48 |
S | - | 0.76 | 0.31 | 0.58 |
Cl | - | 0.61 | - | - |
Proximate | ||||
Moisture | 8.3 ± 0.5 | 8 | - | - |
Volatile matter | 58.6 ± 0.7 | 59.98 | 68.61 | 52.85 |
Fixed carbon | 16.8 ± 0.3 | 12.79 | 15.31 | 14.83 |
Ash | 24.6 ± 0.6 | 19.23 | 16.08 | 32.32 |
Animal manure usually has a high organic and low cellulose content due to bacterial and chemical reactivity in the digestive tract of animals. Bacteria present in the digestive tract of animals help decompose organic matter, such as food residues and other organic matter, which produces manure with a high organic matter content. The digestive process in the animal's body also involves chemical processes that can change the composition of organic matter, including cellulose, into a more easily decomposed form with a high organic matter content and low cellulose levels [13]. Regarding physical and chemical properties, sheep manure contains 15.31–16.67% cellulose, 14.05–26.12% hemicellulose, 13.97–15.21% lignin and pH 6.98–8.52 [6]. Cellulose, hemicellulose and lignin are organic components found in sheep manure that determine the ratio of biomass degradation. A high hemicellulose degradation ratio can increase biogas yield [38], while lignin is more difficult to degrade [39]. In addition to affecting the degradation ability, the content of these organic components also affects the combustion process. Biomass with a high lignin content is more suitable for direct combustion with sufficient oxygen supply, while biomass with a higher cellulose content can be more effective in direct combustion [40]. Biomass derived from plant waste typically has a higher lignocellulosic (lignin and cellulose) content, around 20–50% [41].
One can see in the results of previous studies shown in Table 1 that there is a difference in the percentage of sheep manure component content, but the magnitude is not significant. This difference in content is caused by several factors, namely type of feed and environmental factors [42]. Cotana et al. investigated poultry manure, and the environment during the experiment also influenced the analysis results [43]. Compared to other livestock, sheep manure has the highest fixed carbon content compared to cow, goat, poultry and pig manure, which is 13%, so it has the potential for a high HHV value [6].
Kinetic parameters on sheep manure were analysed using TGA. The mass degradation curve of the sheep manure sample exhibits two distinct stages: dehydration and devolatilisation, as depicted in Figure 1. Dehydration involves the removal of moisture from the biomass. Before the pyrolysis stage, sheep manure biomass undergoes a drying and dehydration process within a temperature range of 30–140°C (Stage 1). During this stage, heat is absorbed by the sheep manure biomass for water evaporation. In the devolatilisation stage (Stage 2 and Stage 3), the gas components within the biomass are separated. Water and light molecules, such as CO and CO2, are released during this stage. The devolatilisation occurs at a temperature range of 210 °C–900 °C. Specifically, Stage 2 (210 °C–500 °C) involves the decomposition of hemicellulose and cellulose, while Stage 3 (>590 °C) involves the degradation of lignin. The decomposition of hemicellulose occurs at temperatures ranging from 220 °C–300 °C, cellulose decomposes at 300 °C–340 °C, and lignin degradation occurs at temperatures exceeding 340 °C [13]. Mishra et al. have also concluded that in biomass pyrolysis in the temperature zone of 150 °C–500 °C, the peak observed was due to the degradation of cellulose and hemicellulose compounds [45]. The Derivative Thermogravimetry (DTG) curve in Figure 1 shows that the highest rate of mass decomposition occurs at temperatures of 250 °C and 450 °C, where the devolatilisation process begins to overcome the manure activation energy barrier.
Conversion fraction in sheep manure vs temperature
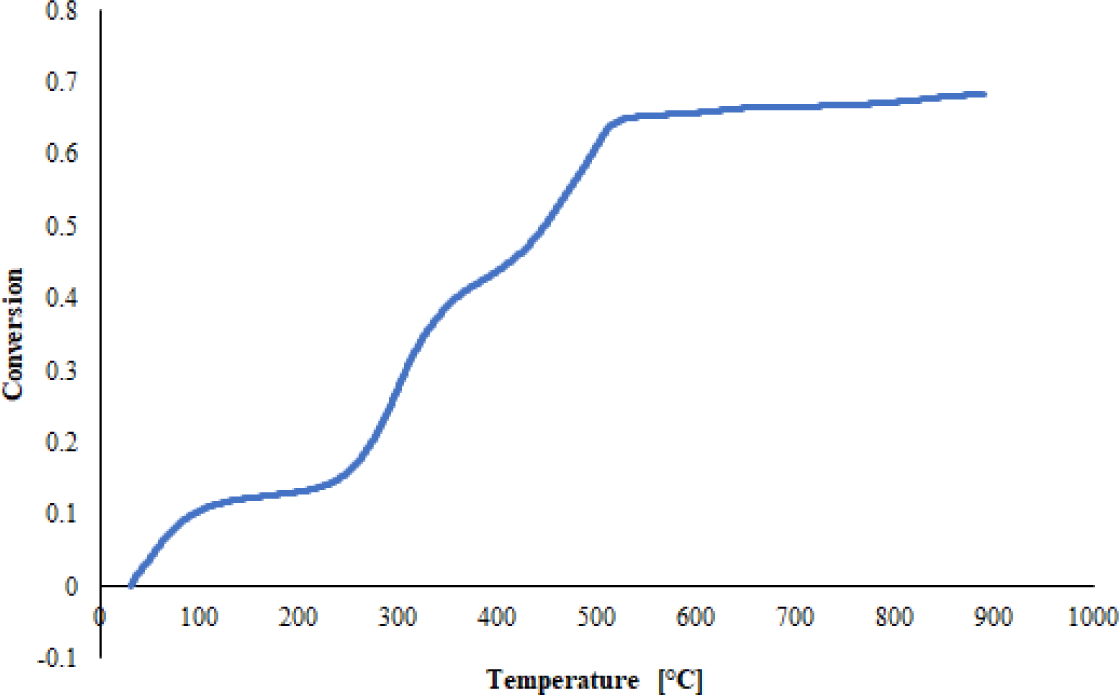
It was reported in previous studies that lignin degradation occurs at temperatures above 450 °C, and the decomposition process typically exhibits a broader temperature range, spanning from 180 °C to 900 °C, with a lower reaction rate [41]. The decomposition curve of hemicellulose, cellulose and lignin in animal manure is influenced by the mineral content of animal manure, namely potassium and sodium [13]. The DTG curve in Figure 1 depicts two peaks in the volatilisation area of hemicellulose and cellulose. This observation contrasts with the research conducted by Chong [13], who reported a single peak in the DTG curve due to horse manure's high potassium and sodium content.
This devolatilisation stage is pyrolysis, in which biomass decomposes in biochar, biogas, and bio-oil products. In the sheep manure DTG curve, sheep manure degrades rapidly and significantly starting at a temperature of 210 °C and will gradually slow down when the temperature is above 500 °C. In the sheep manure DTG curve, there is a rapid and significant degradation starting at a temperature of 210 °C, which gradually slows down when the temperature exceeds 500 °C. After reaching 500 °C, mass degradation steadily decreases until it reaches 900 °C. This observation suggests that most volatile compounds have been lost at this temperature, leaving behind a more stable and less easily decomposable material. The mineral content in animal manure can increase activation energy, thereby influencing carbonisation [46].
Figure 1 depicts about 70% of sheep manure has been degraded at a temperature of 450 °C. At temperatures above 450 °C, lignin degradation continues to a temperature of 900 °C until fully converted. The sheep manure was not completely decomposed to 100%, likely due to the requirement for a TGA test with a heating rate exceeding 10 °C/min. When tested with a heating rate of 10 °C/min, the decomposition of sheep manure reached only approximately 70%. A high heating rate can affect the thermal and mass degradation of the sample [13]. The ash content in sheep manure is 32.32, greater than that of the Rabah [34] study. In addition, ash contains minerals; among these are calcium, potassium, and magnesium found in ash derived from poultry manure [47]. During combustion, the organic matter in the biomass is converted into gases, leaving behind the inorganic minerals as ash [48]. The ash cannot be converted into usable products or energy, and its existence complicates the conversion process. Increasing ash content in biomass can decrease the biomass's volatile matter, carbon, and hydrogen contents, thereby reducing bio-oil yield [49].
Based on the results of TGA testing on sewage sludge by Naqvi et al. [30], the heating rate accelerates the thermal degradation of materials at higher temperatures. This observation suggests that a faster increase in the temperature of sewage sludge samples results in a shorter thermal delay. A higher heating rate also releases a relatively larger amount of volatile substances.
In DSC analysis, changes in heat flow occur when the feedstock changes temperature. The direction of heat flow during the pyrolysis process of sheep manure is shown in Figure 2. Stage 1 is an endothermic reaction in which heat is absorbed, primarily used to evaporate the water content in the biomass. This stage also activates the pyrolysis reaction in Stage 2 by breaking the chemical bonds within the feedstock [50]. Heat flow during pyrolysis increases with rising temperature until it reaches approximately 500 °C. The heat inputs in the reaction at points a, b, and c are respectively −1.00 W·s/mg, 153 mW s/mg, 351 mW·s/mg. Beyond 500 °C, the heat input gradually decreases. Stage 3 is dominated by exothermic reactions where heat is released, and the heat input value decreases further with rising temperature. The exothermic peak in sheep manure is 800 °C in Stage 3, where the heat input value is −140 mW·s/mg.
DSC Curve
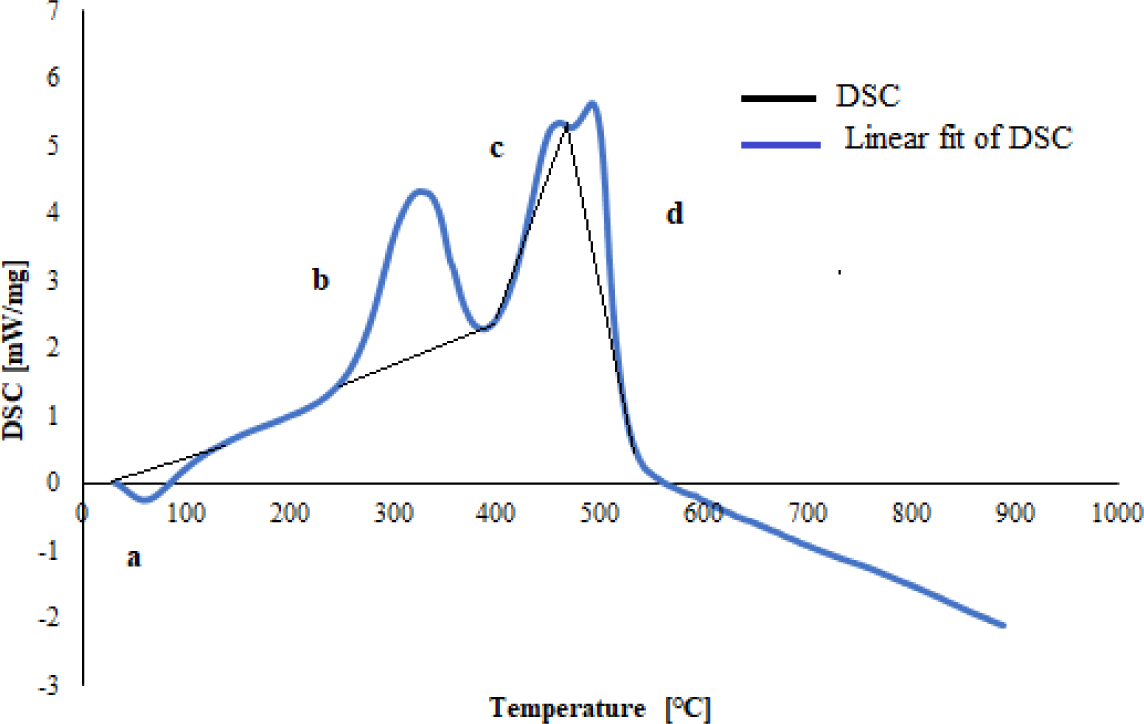
The knowledge of thermal behaviour and reaction kinetics is very important in analysing and developing the pyrolysis process because it can be used to predict reaction rates, optimise experiments and determine operational parameters. In general, the kinetics of pyrolysis is stated in the Arrhenius equation, which involves activation energy, frequency factor and reaction order [51]. Son Hu et al. have also applied the Arrhenius equation to investigate the kinetics of biomass pyrolysis using the Ozawa method [52]. The Friedman model is more widely used than other isoconventional models (Flynn-Wall-Ozawa, Kissinger-Akahira-Sunose, and Staring) because the value of activation energy E calculated by the Friedman model is more accurate and close to the actual value in the pyrolysis process [14]. Friedman's model does not use approximations and assumptions of the Flynn-Wall-Ozawa, Kissinger-Akahira-Sunose, and Staring models. The results of modelling the kinetics of the sheep manure reaction are shown in Figure 3.
Curves of dX/dt vs. 1/T according to Friedman (left) and Coats-Redfern (right)
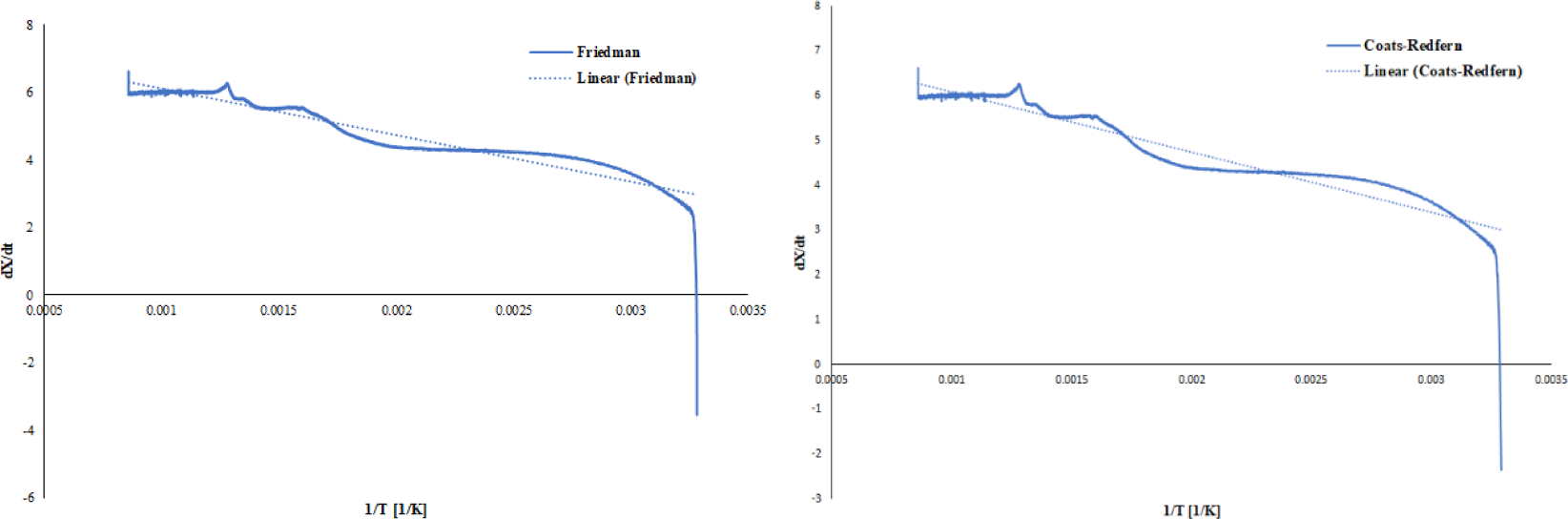
The value of activation energy E can be obtained from plotting data y with x. The value of E is calculated from the slope equation b, and the value of the frequency factor A is obtained from the intercept a. From the graph of the relationship between ln y and x, the R2 value is 0.893 (89.3%). The R2 value indicates that the linear regression model represents the variability of the data. The closer the R2 value is to 1 (100%), the better the linear regression model and vice versa. The graph shows a fairly good R2; thus, the linear regression model can be used. Based on the Arrhenius equation, it is shown that the smaller the value of the frequency factor A, the greater the activation energy E. The frequency factor represents the magnitude of the collisions between reacting molecules. Thus, if the value of the frequency factor is small, it implies that a significant activation energy is required for the reaction to occur. In the context of this pyrolysis study, the decomposition reaction necessitates a higher activation energy when the frequency factor is smaller.
The activation energy required in the pyrolysis process of sheep manure is shown in Figure 4. Activation energy is one of the challenges that must be completed before a chemical reaction occurs. The higher the value of the activation energy, the more complex and difficult a reaction can occur. The activation energy determines the reactivity and rate sensitivity of the reaction [53]. It is possible that the minerals in the manure act as barriers to heat diffusion and release of volatiles that are degraded in the combustion stage. Therefore, the high mineral content in animal waste causes a high activation energy requirement [14]. Overall, the average activation energy of sheep manure based on the Friedman model is 21.32 kJ/mol, and Coats-Redfern is 26.20 kJ/mol.
Activation energy according to Friedman model (left) and Coats-Redfern (right)
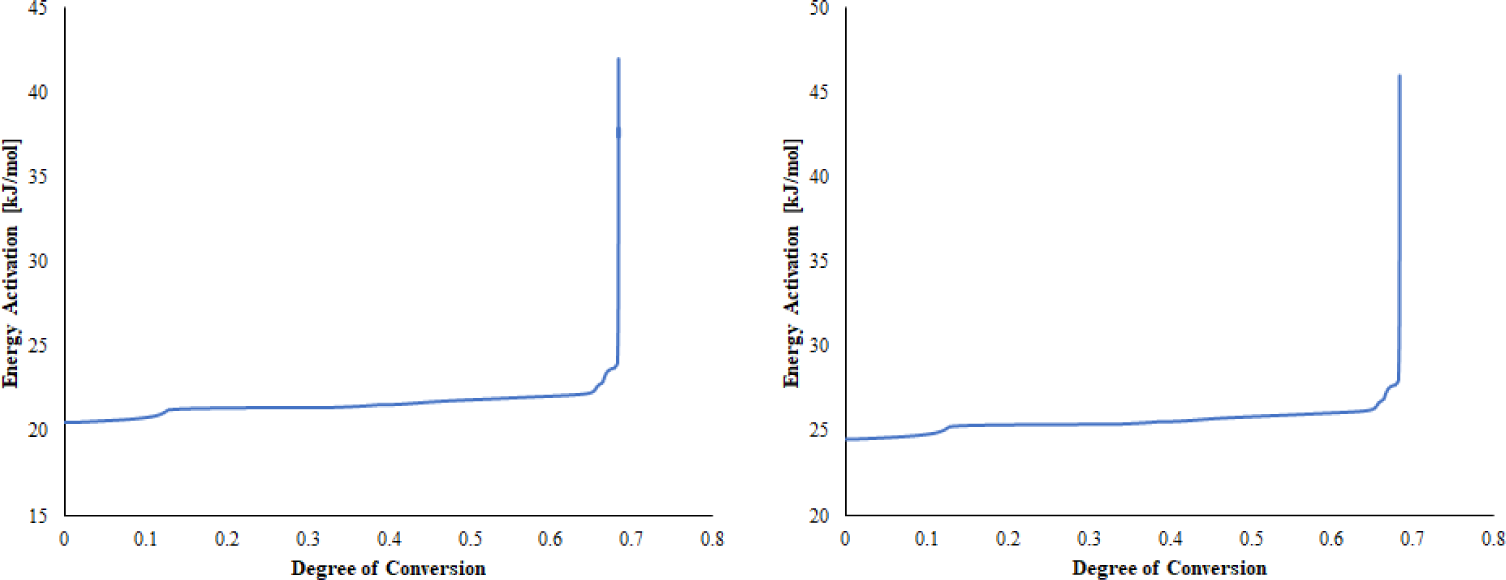
The Friedman and Coats-Redfern modelling shows that the value of the activation energy during the degradation process has increased. At the beginning of the reaction (stage 1, temperature of 30 °C–140°C), the required activation energy is still small, namely 21–22 kJ/mol for the Friedman model and 24–25 kJ/mol for the Coats-Redfern model. This result reflects the degradation of some extractives with small molecules, such as fat, ammonium, sugar, and others [54]. In stage 2, there is a gradual increase in the required activation energy, which tends to be stable when the decomposition of hemicellulose and cellulose occurs. At this stage, an activation energy of 22–25 kJ/mol for the Friedman model is required; for the Coats-Redfern model, it is 25–28 kJ/mol.
The highest increase in activation energy occurs at the degree of conversion 0.65–0.70, which is observed in stage 3. At this stage, lignin decomposition occurs at temperatures above 450 °C. The structure of lignin contains a complex and diverse range of phenolic polymers, which are linked by various strong chemical bonds, including aromatic bonds, ester bonds, and ether bonds. These bonds provide structural strength and resistance to lignin decomposition. Consequently, the activation energy required at this stage is 25–41 kJ/mol for the Friedman model and 28–46 kJ/mol for the Coats-Redfern model.
The results of calculating the thermodynamic parameters are shown in Table 2. The calculation uses equations (10), (11), and (13) to obtain the value of the enthalpy change, Gibbs free energy and entropy change. The value of frequency of factor A is obtained from eq. (12) at the peak temperature of 300 °C with a heating rate of 10 °C/min. The value of A shows the reaction type. If it is small, namely <10−9 1/s, then the reaction that occurs is a surface reaction [55]. However, if the reaction is independent of surface area, the small frequency factor may be due to a closed junctional complex. Conversely, a high value of frequency factor (≥10-9 1/s) indicates the presence of a liberal junctional complex [16]. This interpretation relates to the concepts of surface reactions and junctional complexes. In surface reactions, the chemical reaction occurs at the material's surface, and the low A value indicates the presence of a kinetic step involving adsorption or desorption on a confined surface. Therefore, the low value of the frequency factor indicates a dominant surface reaction in the decomposition of animal manure. However, if the chemical reaction is independent of a small surface area and frequency factor, this could indicate the presence of closed junctional complexes. In other words, chemical reactions occur within the molecular structure or a confined space. Closed junctional complexes can limit the access of reactant molecules and produce a low A value. On the other hand, a high value of the frequency factor may indicate the presence of liberal junctional complexes, where chemical reactions can occur with easier access to the reactant molecules. Liberal junctional complexes are generally open and allow freer interaction of the reactant molecules, which results in a higher frequency factor.
Comparison of thermodynamic parameters in the pyrolysis of animal manure
Manure | Model | A [1/s] | ΔH [kJ/mol] | ΔS [J/mol] | ΔG [kJ/mol] | Reference |
---|---|---|---|---|---|---|
Horse | Friedman | 3.6×1020 | 187.10 | 0.060 | 153.10 | [13] |
Cattle | Friedman | 7.83×1028 | 190.60 | 0.045 | 164.47 | [14] |
Sheep | Friedman | 9.94×1018 | 208.43 | 0.105 | 148.00 | Current work |
Coats-Redfern | 3.42×1018 | 257.32 | 0.192 | 147.00 |
Activation energies differ for different kinds of biomass. An estimate of the energy needed for pyrolysis is provided by the activation energy, which also indicates the energy needed for the decomposition phase. By determining the activation energy, we can know how much the pyrolysis process is influenced by temperature and can optimise the reaction conditions to achieve the desired results. Higher activation energy values might necessitate higher temperatures or more intense reaction conditions, whereas lower values might suggest that the pyrolysis reaction proceeds more readily at a given temperature. Sheep manure has a lower activation energy than many other biomass types, as indicated in Table 3, which shows that pine wood has the lowest activation energy among the materials shown.
Activation energies obtained by this study with those from the literature
Material | Activation energy [kJ/mol] | Method | Reference |
---|---|---|---|
Cotton husk | 187.42–269.09 | Distributed Activation Energy Model (DAEM) | [56] |
Sugarcane trash | 135.07–320.00 | DAEM | [57] |
Municipal solid waste | 13.00–42.00 | Coats and Redfern | [58] |
Plastic | 81.00–140.00 | Kissinger-Akahira-Sunnose (KAS) | [59] |
Pine wood | 11.66–14.08 | Friedman | [60] |
Alga polysiphonia elongata | 71.11–147.57 | Friedman | [61] |
Chicken manure | 112.10 87.64–89.96 |
Flynn-Wall-Ozawa (FWO) Coats-Redfern |
[10] |
Camel manure | 100.06–150.79 | Coats-Redfern | [16] |
Sheep manure | 21.32 26.20 200.2 |
Friedman Coats-Redfern KAS |
Current work |
Horse manure | 194.6 199.3 129.67–348.27 |
Friedman FWO Friedman |
[13] |
Cattle manure |
121.73–327.19 119.40–332.39 140.32 |
FWO KAS FWO |
[14] |
Cow manure |
152.38 138.82 135.20 |
Friedman KAS FWO |
[18] |
Buffalo manure |
143.33 134.68 184.70 |
Friedman KAS FWO |
[18] |
Swine manure | 181.34 181.73 |
KAS Starink |
[62] |
A positive ΔH indicates that heat must be supplied from an external source for the pyrolysis process to occur. In this study, pyrolysis requires additional heat to activate and continue the chemical reactions taking place in the feedstock sample. The higher the ΔH value, the greater the amount of heat required in the pyrolysis process. In the table, all animal manure has a positive enthalpy change value, indicating that the pyrolysis process requires heat to start the reaction. In a study conducted by Parthasarathy et al., it was concluded that the ΔH values at conversion degrees of 0.7 and 0.8 reached the highest values. This indicates that at this conversion fraction, the pyrolysis process requires more heat to continue the chemical reaction in the feedstock sample [21].
The difference between activation energy and enthalpy in sheep manure is quite large, where activation energy has a smaller value than enthalpy. This observation indicates that sheep manure's chemical reactions or thermal processes tend to start more easily and proceed more quickly. It can be a favourable condition for optimising bioenergy production from sheep manure. In the pyrolysis process of sheep manure, the low activation energy allows the degradation of raw materials to start easily so that the reaction takes place more quickly. A higher reaction speed means more biochar or bio-oil products can be produced in a shorter time. However, based on the conversion vs temperature curve in Figure 4, the mass decomposition of sheep manure with a heating rate of 10°C/min can only decompose raw materials up to 70%. Therefore, one should consider heating rates above 10 °C/min because at lower rates, it takes longer, and a higher temperature (> 900 °C) is required to decompose the manure up to 80–90%.
The Gibbs free energy change (ΔG) is a thermodynamic potential that reflects the balance between enthalpy (H) and entropy (S) in a system and provides valuable information about the favourability and direction of a chemical reaction. In pyrolysis reactions, ΔG is important for understanding chemical reactions that occur, predicting reaction directions, favourability of a reaction's thermodynamics, energy changes, system stability, and thermodynamic calculations [63]. A positive ΔG value indicates that the system's free energy increases during the reaction, i.e., external energy is required to overcome the activation energy barrier, start the reaction, and keep it running [21]. Based on Table 2, the ΔG value for all animal wastes is positive, indicating that manure pyrolysis is an endothermic reaction that requires energy to start.
Entropy change (ΔS) can be used as an indicator to understand the level of reactivity of feedstock samples during the pyrolysis process. A high ΔS indicates high reactivity, while a low ΔS indicates low reactivity. A conversion fraction of 0.7 often indicates maximum reactivity, while a conversion fraction 0.1 indicates low reactivity [21]. Increased S during the pyrolysis process indicates that the feedstock transforms into biochar. Conversely, decreased S would indicate that the amount of unreacted sample material increases during pyrolysis [64]. For all conversion fractions, only low ΔS values were found, indicating that the pyrolysis process runs close to thermodynamic equilibrium [65]. In other words, the pyrolysis reaction approaches a system's state where no significant change in the system's entropy can occur.
The findings of the physicochemical, kinetic and thermodynamic studies showed that sheep manure is a valuable source of bioenergy and has good potential to be used as feedstock for pyrolysis because it contains the highest C and H percentages compared to other livestock manure. TGA of sheep manure was conducted with a heating rate 10 °C/min in a temperature range of 30 °C–900°C. Based on the results, the stages of dehydration and devolatilisation were identified. The temperature range for the dehydration is 30 °C–140 °C, while the devolatilisation is 210 °C–900 °C. The devolatilisation stage includes the degradation of cellulose and hemicellulose (210 °C–500 °C) and lignin (>590 °C). However, at temperatures above 500 °C, mass degradation decreases until 900 °C is reached. At this temperature, volatile compounds are no longer present in the solid phase because they have been released into the gas phase.
The analysis of pyrolysis reaction kinetics utilised the Friedman model. It resulted in an average activation energy of 21.32 kJ/mol, while the Coats-Redfern model yielded 26.20 kJ/mol. The calculated activation energy is used to estimate thermodynamic parameters such as entropy, enthalpy, and Gibbs free energy. The frequency factor obtained is 9.94×1018, which indicates that the pyrolysis process of sheep manure is complicated. The reaction enthalpy value of 208.43 kJ/mol (Friedman) or 257.32 kJ/mol (Coats-Redfern) indicates that pyrolysis of sheep manure is endothermic. The high entropy change indicates that the pyrolysis of sheep manure has high reactivity.
The study of TGA, kinetics and thermodynamics is one of the basics of developing pyrolysis reactors, starting with fabrication design and configuration. Based on thermodynamic analysis, the difference between activation energy and enthalpy in sheep manure is quite large, where activation energy has a smaller value than enthalpy. This observation indicates that sheep manure's chemical reactions or thermal processes start more easily and proceed more quickly. However, according to the conversion curve, the decomposition of sheep manure mass with a heating rate of 10°C/min can only reach up to 70%. For further studies on optimising the pyrolysis process, it is necessary to test the pyrolysis with a heating rate above 10 °C/min because, at a low heating rate, it takes a longer time and a higher temperature (> 900°C) to decompose the manure up to 80–90%.
This research is funded by the Directorate of Research and Development, Universitas Indonesia, under Seed Funding: Hibah Profesor dan Lektor Kepala FTUI 2023.
A | frequency factor | [1/s] |
rate of conversion | [kg/s] | |
E | activation energy | [kJ/mol] |
f(X) | kinetic function of the reaction mechanism | [-] |
G | Gibbs free energy | [kJ/mol] |
H | enthalpy | [kJ/mol] |
h | Planck constant | [Js] |
k | rate constant for first-order reaction | [1/s] |
kB | Boltzmann constant | [J/K] |
R | universal gas constant | [kJ/mol K] |
S | entropy | [J/mol] |
T | temperature | [°C] or [K] |
TP | temperature at the peak of sample decomposition | [K] |
X | conversion fraction | [-] |
β | heating rate | [°C/s] or [K/s] |
DAEM | Distributed Activation Energy Model |
DSC | Differential Scanning Calorimetry Analysis |
DT | Derivative Thermogravimetry |
DTA | Differential Thermal Analysis |
FWO | Flynn-Wall-Ozawa |
KAS | Kissinger-Akahira-Sunnose |
NOAA | National Oceanic and Atmospheric Administration |
SDG | Sustainable Development Goal |
TG | Thermogravimetry |
TGA | Thermogravimetric Analysis |
A critical review on livestock manure biorefinery technologies: Sustainability, challenges, and future perspectives ,Renew. Sustain. Energy Rev. , Vol. 135 , 2021, https://doi.org/https://doi.org/10.1016/j.rser.2020.110033
, A review on biomass and wind as renewable energy for sustainable environment ,Chemosphere , Vol. 293 ,pp 133579 , 2022, https://doi.org/https://doi.org/10.1016/j.chemosphere.2022.133579
, Handbook of Energy and Economic Statistics of Indonesia , 20212023
, Pyrolysis of goat manure to produce bio-oil ,Eng. Sci. Technol. an Int. J. , Vol. 22 (2),pp 452–457 , 2019, https://doi.org/https://doi.org/10.1016/j.jestch.2018.11.002
, - Livestock in Figures 2022, 20222023, https://www.bps.go.id/en/publication/2022/06/30/4c014349ef2008bea02f4349/peternakan-dalam-angka-2022.html., Accessed
A review on prominent animal and municipal wastes as potential feedstocks for solar pyrolysis for biochar production ,Fuel , Vol. 316 , 2022, https://doi.org/https://doi.org/10.1016/j.fuel.2022.123378
, Pyrolysis of lignocellulosic, algal, plastic, and other biomass wastes for biofuel production and circular bioeconomy: A review of thermogravimetric analysis (TGA) approach ,Renew. Sustain. Energy Rev. , Vol. 169 , 2022, https://doi.org/https://doi.org/10.1016/j.rser.2022.112914
, Mechanistic investigation of pyrolysis kinetics of water hyacinth for biofuel employing isoconversional method ,Sustain. Energy Technol. Assessments , Vol. 57 , 2023, https://doi.org/https://doi.org/10.1016/j.seta.2023.103175
, Pyrolysis of Waste Castor Seed Cake: A Thermo-Kinetics Study ,Eur. J. Sustain. Dev. Res. , Vol. 2 (2), 2018, https://doi.org/https://doi.org/10.20897/ejosdr/81642
, Pyrolysis and optimisation of chicken manure wastes in fluidised bed reactor: CO2 capture in activated bio-chars ,Process Safety and Environ. Protection. , Vol. 130 ,pp 297-305 , 2019, https://doi.org/https://doi.org/10.1016/j.psep.2019.08.011
, Thermogravimetric kinetics of lignocellulosic biomass slow pyrolysis using distributed activation energy model, Fraser–Suzuki deconvolution, and iso-conversional method ,Energy Convers. Manag. , Vol. 118 ,pp 1–11 , 2016, https://doi.org/https://doi.org/10.1016/j.enconman.2016.03.058
, - , Biomass Gasification and Pyrolysis Practical Design, 2010
Pyrolysis characteristics and kinetic studies of horse manure using thermogravimetric analysis ,Energy Convers. Manag. , Vol. 180 ,pp 1260–1267 , 2019, https://doi.org/https://doi.org/10.1016/j.enconman.2018.11.071
, Cattle manure pyrolysis process: Kinetic and thermodynamic analysis with isoconversional methods ,Renew. Energy , Vol. 107 ,pp 489–496 , 2017, https://doi.org/https://doi.org/10.1016/j.renene.2017.02.026
, Thermal Decomposition Characteristics and Kinetic Analysis of Chicken Manure in Various Atmospheres ,Agriculture , Vol. 12 (5), 2022, https://doi.org/https://doi.org/10.3390/agriculture12050607
, Thermal degradation characteristics and gasification kinetics of camel manure using thermogravimetric analysis ,J Env. Manag. , Vol. 287 ,pp 112345 , 2021, https://doi.org/https://doi.org/10.1016/j.jenvman.2021.112345
, Effect of heating rate on the pyrolysis of camel manure ,Biomass Convers. Biorefinery , Vol. 13 (7),pp 6023–6035 , 2021, https://doi.org/https://doi.org/10.1007/s13399-021-01531-9
, Non-isothermal thermo-kinetics and empirical modeling: Comparative pyrolysis of cow and Buffalo manure ,Therm. Sci. Eng. Prog. , Vol. 37 , 20222023, https://doi.org/https://doi.org/10.1016/j.tsep.2022.101568
, P Effects of pyrolysis temperature and reaction time on the performance of swine-manure-derived bio-binder ,Transportation Research Part D: Transport and Environment , Vol. 89 , 2020, https://doi.org/https://doi.org/10.1016/j.trd.2020.102608
, Kinetic analysis of manure pyrolysis and combustion processes ,Waste Manag. , Vol. 58 ,pp 230–240 , 2016, https://doi.org/https://doi.org/10.1016/j.wasman.2016.08.027
, Thermogravimetric analysis of camel dung, date stone, and their blend for pyrolytic, kinetic, and thermodynamic studies ,Clean. Chem. Eng. , Vol. 4 ,pp 100072 , 2022, https://doi.org/https://doi.org/10.1016/j.clce.2022.100072
, Pyrolysis of dairy cattle manure: evolution of char characteristics ,J. Anal. Appl. Pyrolysis , Vol. 145 ,pp 104724 , 2020, https://doi.org/https://doi.org/10.1016/j.jaap.2019.104724
, Synergetic effects during co-pyrolysis of sheep manure and recycled polyethylene terephthalate ,Polymers (Basel). , Vol. 13 (14), 2021, https://doi.org/https://doi.org/10.3390/polym13142363
, Recovery of clean energy precursors from Bambara groundnut waste via pyrolysis: Kinetics, products distribution and optimisation using response surface methodology ,J. Clean. Prod. , Vol. 164 ,pp 1430–1445 , 2017, https://doi.org/https://doi.org/10.1016/j.jclepro.2017.07.068
, A hybrid kinetic and optimisation approach for biomass pyrolysis: The hybrid scheme of the isoconversional methods, DAEM, and a parallel-reaction mechanism ,Energy Convers. Manag. , Vol. 208 , 2020, https://doi.org/https://doi.org/10.1016/j.enconman.2020.112531
, Processing of TGA data: Analysis of isoconversional and model fitting methods ,Fuel , Vol. 165 ,pp 490–498 , 2016, https://doi.org/https://doi.org/10.1016/j.fuel.2015.10.042
, Pseudo-component thermal decomposition kinetics of tomato peels via isoconversional methods ,Fuel Process. Technol. , Vol. 154 ,pp 243–250 , 2016, https://doi.org/https://doi.org/10.1016/j.fuproc.2016.09.001
, Pyrolysis of Grape Marc from Tunisian Wine Industry: Feedstock Characterization, Thermal Degradation and Kinetic Analysis ,Energies , Vol. 11 (4), 2018, https://doi.org/https://doi.org/10.3390/en11040730
, Comparison of kinetic analysis methods in thermal decomposition of cattle manure by themogravimetric analysis ,Bioresour. Technol. , Vol. 243 ,pp 69–77 , 2017, https://doi.org/https://doi.org/10.1016/j.biortech.2017.06.007
, Pyrolysis of high ash sewage sludge: Kinetics and thermodynamic analysis using Coats-Redfern method ,Renew. Energy , Vol. 131 ,pp 854–860 , 2019, https://doi.org/https://doi.org/10.1016/j.renene.2018.07.094
, Thermal degradation characteristics and kinetic study of camel manure pyrolysis ,J. Environ. Chem. Eng. , Vol. 9 (5), 2021, https://doi.org/https://doi.org/10.1016/j.jece.2021.106071
, Understanding pyrolysis mechanisms of corn and cotton stalks via kinetics and thermodynamics ,J. Anal. Appl. Pyrolysis , Vol. 164 ,pp 105521 , 2022, https://doi.org/https://doi.org/10.1016/j.jaap.2022.105521
, Enthalpy for Pyrolysis for Several Types of Biomass ,Energy&Fuels , Vol. 17 ,pp 934–939 , 2003, https://doi.org/https://doi.org/10.1021/ef020260x
, Livestock manure availability and syngas production: A case of Sudan ,Energy , Vol. 259 ,pp 124980 , 2022, https://doi.org/https://doi.org/10.1016/j.energy.2022.124980
, The impacts of biomass properties on pyrolysis yields, economic and environmental performance of the pyrolysis-bioenergy-biochar platform to carbon negative energy ,Bioresour Technol , Vol. 241 ,pp 959–968 , 2017, https://doi.org/https://doi.org/10.1016/j.biortech.2017.06.049
, Slow Pyrolysis of Oil Palm Empty Fruit Bunches for Biochar Production and Characterisation ,J. Phys. Sci. , Vol. 25 (2),pp 97–112 , 2014
, - , Introduction, Torrefaction of Biomass for Energy Applications, 2018
Pretreatment of Animal Manure Biomass to Improve Biogas Production: A Review ,Energies , Vol. 13 (14), 2020, https://doi.org/https://doi.org/10.3390/en13143573
, Horse Manure and Lignocellulosic Biomass Characterization as Methane Production Substrates ,Fermentation , Vol. 9 (6), 2023, https://doi.org/https://doi.org/10.3390/fermentation9060580
, Effects of Hemicellulose and Lignin on Enzymatic Hydrolysis of Cellulose from Dairy Manure ,Appl. Biochem. Biotechnol. , Vol. 121-1 ,pp 1017–1030 , 2005, https://doi.org/https://doi.org/10.1385/ABAB:124:1-3:1017
, Kinetic Parameters Determination of Biomass Pyrolysis Fuels Using TGA and DTA Techniques ,Waste and Biomass Valorization , Vol. 6 (3),pp 401–415 , 2015, https://doi.org/https://doi.org/10.1007/s12649-015-9354-7
, Biochar physicochemical properties: pyrolysis temperature and feedstock kind effects ,Rev. Environ. Sci. Bio/Technology , Vol. 19 (1),pp 191–215 , 2020, https://doi.org/https://doi.org/10.1007/s11157-020-09523-3
, Energy Valorization of Poultry Manure in a Thermal Power Plant: Experimental Campaign ,Energy Procedia , Vol. 45 ,pp 315–322 , 2014, https://doi.org/https://doi.org/10.1016/j.egypro.2014.01.034
, Feedstock Dependence of Emissions from a Reverse-Downdraft Gasifier Cookstove ,Energy Sustain. Dev. , Vol. 56 ,pp 42–50 , 2020, https://doi.org/https://doi.org/10.1016/j.esd.2020.02.008
, Pyrolysis kinetics and thermal behavior of waste sawdust biomass using thermogravimetric analysis ,Bioresour. Technol. , Vol. 251 ,pp 63–74 , 2018, https://doi.org/https://doi.org/10.1016/j.biortech.2017.12.029
, Investigation of thermodynamic parameters in the pyrolysis conversion of biomass and manure to biochars using thermogravimetric analysis ,Bioresour. Technol. , Vol. 146 ,pp 485–493 , 2013, https://doi.org/https://doi.org/10.1016/j.biortech.2013.07.086
, Ash 0086from Poultry Manure Incineration as a Substitute for Phosphorus Fertiliser ,Materials (Basel). , Vol. 15 (9), 2022, https://doi.org/https://doi.org/10.3390/ma15093023
, Ash formation and deposition in coal and biomass fired combustion systems: Progress and challenges in the field of ash particle sticking and rebound behavior ,Prog. energy Combust. Sci. , Vol. 68 ,pp 65–168 , 2018, https://doi.org/https://doi.org/10.1016/j.pecs.2018.02.001
, - ,
Critical Analysis of Process Parameters for Bio-oil Production via Pyrolysis of Biomass: A Review ,Recent Patents Eng. , Vol. 7 (2),pp 98–114 , 2013, https://doi.org/https://doi.org/10.2174/18722121113079990005 Fundamentals, kinetics and endothermicity of the biomass pyrolysis reaction ,Renew. Energy , Vol. 35 (1),pp 232–242 , 2010, https://doi.org/https://doi.org/10.1016/j.renene.2009.04.019
, Pyrolysis characteristics and kinetics of microalgae via thermogravimetric analysis (TGA): A state-of-the-art review ,Bioresour Technol , Vol. 246 ,pp 88–100 , 2017, https://doi.org/https://doi.org/10.1016/j.biortech.2017.06.087
, Kinetic study of Chinese biomass slow pyrolysis: Comparison of different kinetic models ,Fuel , Vol. 86 (17–18),pp 2778–2788 , 2007, https://doi.org/https://doi.org/10.1016/j.fuel.2007.02.031
, Pyrolysis of olive residue and sugar cane bagasse: Non-isothermal thermogravimetric kinetic analysis ,Bioresour. Technol. , Vol. 102 (24),pp 11234–11238 , 2011, https://doi.org/https://doi.org/10.1016/j.biortech.2011.09.010
, Investigation of thermodynamic parameters in the pyrolysis conversion of biomass and manure to biochars using thermogravimetric analysis ,Bioresour Technol , Vol. 146 ,pp 485–493 , 2013, https://doi.org/https://doi.org/10.1016/j.biortech.2013.07.086
, Kinetic parameters of red pepper waste as biomass to solid biofuel ,Bioresour. Technol. , Vol. 204 ,pp 157–163 , 2016, https://doi.org/https://doi.org/10.1016/j.biortech.2015.12.055
, Kinetic study of solid waste pyrolysis using distributed activation energy model ,Bioresour. Technol. , Vol. 178 ,pp 126–131 , 2015, https://doi.org/https://doi.org/10.1016/j.biortech.2014.10.028
, Characterisation of coal and biomass based on kinetic parameter distributions for pyrolysis ,Fuel , Vol. 114 ,pp 206–215 , 2013, https://doi.org/https://doi.org/10.1016/j.fuel.2012.04.023
, Investigation of (co)-combustion kinetics of biomass, coal and municipal solid wastes ,Waste Manag. , Vol. 97 ,pp 10–18 , 2019, https://doi.org/https://doi.org/10.1016/j.wasman.2019.07.033
, Pyrolysis and combustion kinetics of refuse derived fuel having different plastic ratio ,Bioresour. Technol. Reports , Vol. 23 , 2023, https://doi.org/https://doi.org/10.1016/j.biteb.2023.101559
, A combined analysis of the drying and decomposition kinetics of wood pyrolysis using non-isothermal thermogravimetric methods ,Energy Convers. Manag. X , Vol. 20 , 2023, https://doi.org/https://doi.org/10.1016/j.ecmx.2023.100424
, Thermal behaviour and kinetics of alga Polysiphonia elongata biomass during pyrolysis ,Bioresour. Technol. , Vol. 171 (1),pp 193–198 , 2014, https://doi.org/https://doi.org/10.1016/j.biortech.2014.08.064
, Insights into the kinetics, thermodynamics and evolved gases for the pyrolysis of freshly excreted and solid-liquid separated swine manures ,Energy , Vol. 288 ,pp 129855 , 2024, https://doi.org/https://doi.org/10.1016/j.energy.2023.129855
, Kinetic and thermodynamic analyses of dried oily sludge pyrolysis ,J. Energy Inst. , Vol. 95 ,pp 30–40 , 2021, https://doi.org/https://doi.org/10.1016/j.joei.2020.12.002
, - , Chapter 2 - Kinetic Analysis of Biomass Pyrolysis, 2018
Thermokinetic and TG/DSC-FTIR study of pea waste biomass pyrolysis ,Appl. Therm. Eng. , Vol. 137 ,pp 54–61 , 2018, https://doi.org/https://doi.org/10.1016/j.applthermaleng.2018.03.050.
,